Health Transformation — Management Institute
Joaquim Cardoso MSc*
Chief Strategy Officer, Researcher and Editor
November 9, 2022
(*MSC from London Business School — MIT Sloan Masters Program)
Source: Nature Reviews Materials
Executive Summary:
- Wearable devices provide an alternative pathway to clinical diagnostics by exploiting various physical, chemical and biological sensors to mine physiological (biophysical and/or biochemical) information in real time (preferably, continuously) and in a non-invasive or minimally invasive manner.
- These sensors can be worn in the form of glasses, jewellery, face masks, wristwatches, fitness bands, tattoo-like devices, bandages or other patches, and textiles.
- Wearables such as smartwatches have already proved their capability for the early detection and monitoring of the progression and treatment of various diseases, such as COVID-19 and Parkinson disease, through biophysical signals.
- Next-generation wearable sensors that enable the multimodal and/or multiplexed measurement of physical parameters and biochemical markers in real time and continuously could be a transformative technology for diagnostics, allowing for high-resolution and time-resolved historical recording of the health status of an individual.
- In this Review, we examine the building blocks of such wearable sensors, including the substrate materials, sensing mechanisms, power modules and decision-making units, by reflecting on the recent developments in the materials, engineering and data science of these components.
- Finally, we synthesize current trends in the field to provide predictions for the future trajectory of wearable sensors.
Next-generation wearable sensors that enable the multimodal and/or multiplexed measurement of physical parameters and biochemical markers in real time and continuously could be a transformative technology for diagnostics,
Infographic
Fig. 1: Timeline of major milestones in the development of wearable sensors and a summary of their building blocks.
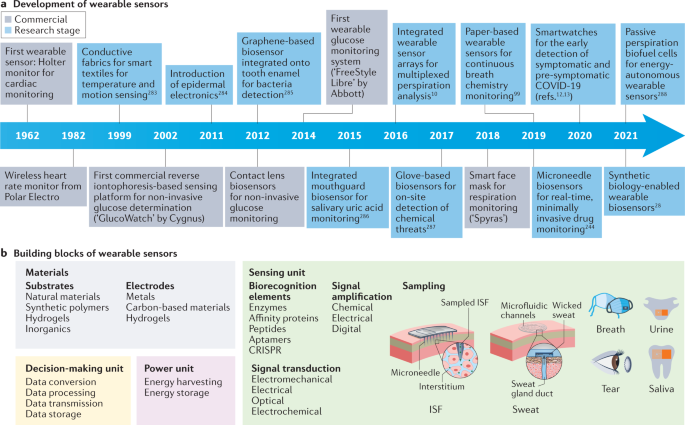
Fig. 2: The decision-making unit and its working principles.
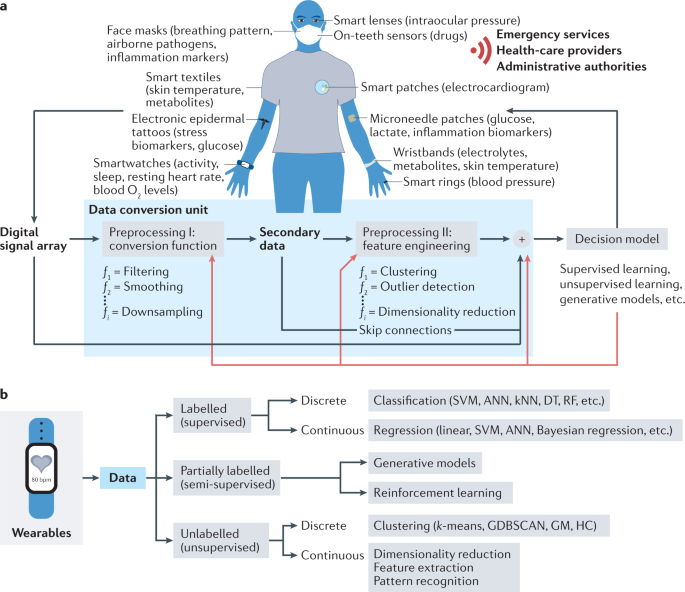
Fig. 2 | The decision-making unit and its working principles.
a | Conceptualization of the data pipeline.
The combination and processing of multiple wearables with multiple sensing strategies provides access to physiologically relevant parameters and biomarkers to better explain the non-linearity in human physiology. The black and red lines indicate the data processing and model training pathways, respectively.
b | Overview of data-driven methods.
Post-processing of big data to explore the complex links between the measured signals and physiological status of individuals is possible with machine learning algorithms. ANN, artificial neural network; DT, decision tree; GDBSCAN, generalized density-based spatial clustering of applications with noise; GM, Gaussian means; HC, hierarchical clustering; kNN, k-nearest neighbours; RF, random forest; SVM, support-vector machines. Panel a (top part) adapted from ref. 14, Springer Nature Limited.
Fig. 3: Energy harvesting methods.
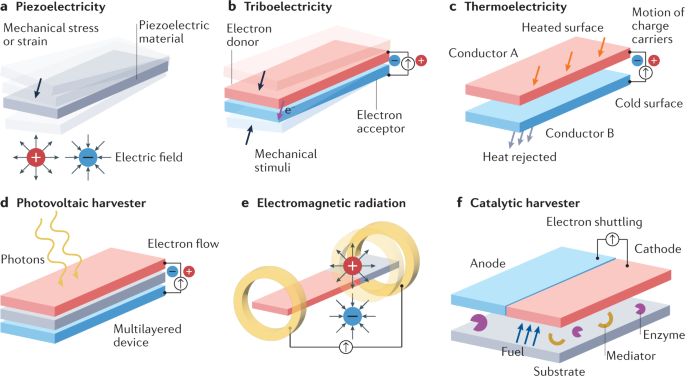
Fig. 3 | Energy harvesting methods.
a | Piezoelectricity is generated by mechanical motion, which activates a piezoelectric material. b | Triboelectricity is produced by motion that results in the physical contact and separation of two materials with different electronegativities. c | Thermoelectricity is generated when the surface of conductor A is heated and this energy is then transferred to conductor B, which triggers the motion of charge carriers (such as electrons and holes) and generates a voltage. d | Photovoltaic energy is generated when a photovoltaic material is irradiated with light. e | Electromagnetic radiation is managed by antennas that transform electromagnetic waves into a voltage or current. f| Wearable biofuel cells create energy from a catalytic reaction, which occurs between the fuel provided by a biofluid (such as sweat) and an enzyme; the reaction is generally enhanced by a mediator that boosts the electron transfer process between the enzymes and the electrodes.
ORIGINAL PUBLICATION (Excerpt version)

End-to-end design of wearable sensors
Nature Reviews Materials
H. Ceren Ates, Peter Q. Nguyen, Laura Gonzalez-Macia, Eden Morales-Narváez, Firat Güder, James J. Collins & Can Dince
Introduction
Wearable sensors are integrated analytical devices that combine typical characteristics of point-of-care systems with mobile connectivity in autonomously operating, self-contained units.
Such devices allow for the continuous monitoring of the biometrics of an individual in a non-invasive or minimally invasive manner, enabling the detection of small physiological changes from baseline values over time1.
Wearables have existed for decades (Fig. 1a); for example, the Holter monitor, a medical sensor used for measuring the electrical activity of the heart, dates back to the 1960s2.
Although the total number of components might vary depending on the specific application, the common building blocks (Fig. 1b) of wearable devices are the substrate and electrode materials, sensing units (elements for interfacing, sampling, biorecognition, signal transduction and amplification), decision-making units (components for data collection, processing and transmission) and power units3.
Fig. 1: Timeline of major milestones in the development of wearable sensors and a summary of their building blocks.
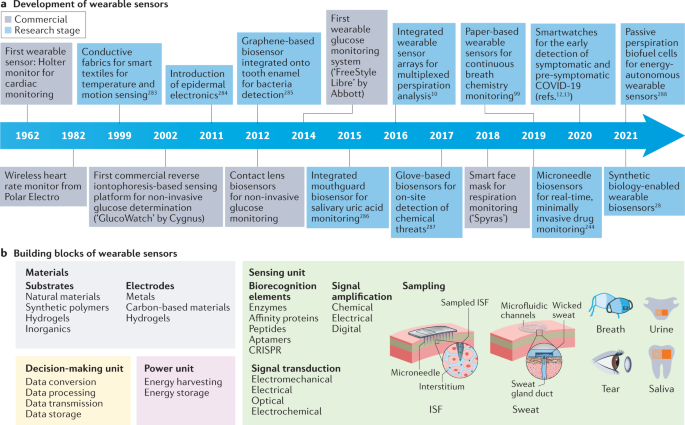
a | Major commercial and research-stage milestones in the development of wearable devices for health-care monitoring 10,12,13,28,99,244,283,284,285,286,287,288.
Advances in telecommunication technologies, materials science, bioengineering, electronics and data analysis, together with the rapidly increasing interest in monitoring health and well-being, have been the primary drivers of innovation in modern wearable sensors148.
More recently, the considerable reductions in cost have enabled the penetration of modern wearable sensors into many segments of the (consumer) population and geographical regions of the world, unlocking continuous monitoring at a scale never seen before.
In addition, advances in fabrication methods have enabled greater sophistication at increasingly smaller dimensions, enabling sensor platforms to reach scales amenable to integration into personal technologies.
b | Building blocks of wearable devices, including the substrate and electrode materials and the components of the sensing, decision-making and power units. ISF, interstitial fluid. Panel b (on-tooth sensor) adapted from ref.285, Springer Nature Limited.
More recently, the considerable reductions in cost have enabled the penetration of modern wearable sensors into many segments of the (consumer) population and geographical regions of the world, unlocking continuous monitoring at a scale never seen before.
In addition, advances in fabrication methods have enabled greater sophistication at increasingly smaller dimensions, enabling sensor platforms to reach scales amenable to integration into personal technologies.
Modern wearables can perform high-quality measurements comparable to those of regulated medical instruments.
Hence, the divide between consumer and medical wearable devices is increasingly blurred.
Modern wearables can perform high-quality measurements comparable to those of regulated medical instruments. Hence, the divide between consumer and medical wearable devices is increasingly blurred.
First-generation wearables, in the form of watches, shoes or headsets, have mainly focused on biophysical monitoring by tracking the physical activity, heart rate or body temperature of an individual1,4,5.
With the wide adoption and success of first-generation wearables, the focus has been slowly shifting towards non-invasive or minimally invasive biochemical and multimodal monitoring, which is the next step in realizing truly individualized health care6,7,8.
First-generation wearables, in the form of watches, shoes or headsets, have mainly focused on biophysical monitoring by tracking the physical activity, heart rate or body temperature of an individual
These second-generation wearables encompass form factors such as on-skin patches, tattoos, tooth-mounted films, contact lenses and textiles, as well as more invasive microneedles and injectable devices9,10,11.
A key characteristic of second-generation wearables is the use of biofluids, whereby biorecognition elements are used to convert the presence of a specific analyte into a detectable signal.
These second-generation wearables encompass form factors such as on-skin patches, tattoos, tooth-mounted films, contact lenses and textiles, as well as more invasive microneedles and injectable devices. A key characteristic …is the use of biofluids…
Most of these examples are laboratory prototypes, but there are some commercial exceptions (including the FreeStyle Libre glucose monitoring system and the Gx Sweat Patch)1.
Most of these examples are laboratory prototypes, but there are some commercial exceptions (including the FreeStyle Libre glucose monitoring system and the Gx Sweat Patch)
Wearable biochemical and biophysical sensors have been used to detect and manage diseases1,12,13,14,15 and for wellness applications16,17,18.
The use of wearable devices, however, extends beyond human-centred health and well-being as their applications have also proliferated in animal health monitoring for the pet and animal husbandry markets19.
Wearable biochemical and biophysical sensors have been used to detect and manage diseases, and for wellness applications
The use of wearable devices, however, extends beyond human-centred health and well-being as their applications have also proliferated in animal health monitoring for the pet and animal husbandry markets
This Review details the recent developments in the field of wearable sensors with a particular focus on the sensing, decision-making and power units to establish a framework for the design and implementation of wearable devices.
As we examine the various building blocks of wearable sensors, we also analyse the current trends, discuss the challenges and provide recommendations to establish a vision for how this field might evolve in the next decade to transform health care.
Other sections
See the original publication (this is an excerpted version only)
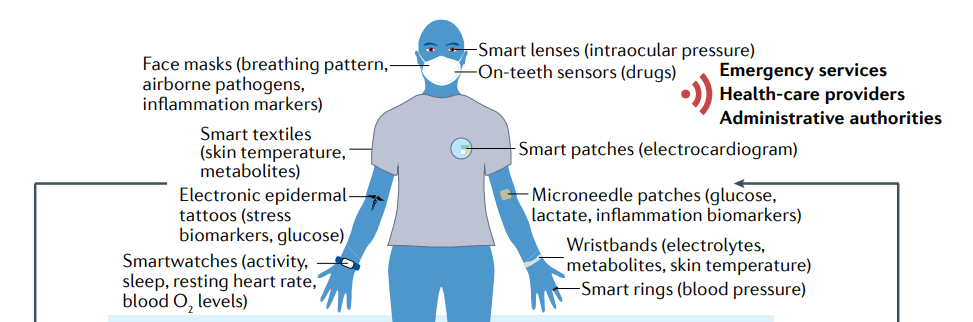
Outlook
With continued innovation and development, together with the widespread use of wearables, we are now many steps closer to fulfilling the prerequisite for proactive health care by monitoring the time-resolved variation in the physiological state of the body.
Yet, there remain numerous challenges and areas for development to realize the full potential of wearable sensing devices.
From the materials perspective, the development of breathable, flexible and stretchable materials (such as superflexible wood214) is still an important challenge to satisfy the rigorous requirements of wearable applications (such as adaptation to electronic skins, smart patches or textiles).
Furthermore, transient and recyclable (even compostable) substrate materials are desired for the sustainable and low-cost mass production of wearable sensors.
Another challenge is to develop self-powered wearables, including ‘green’ power units (such as disposable solar panels or biofuel cells) or powerless options through near-field communication.
These advances could lead to the evolution of standalone, fully integrated wearable sensors, or a biosensing unit that operates in combination with other ubiquitous personal devices (such as smartphones).
To enable robust long-term (days to weeks) continuous measurement in a wearable format, sensing and sampling technologies should be further matured.
In this regard, future trends for advanced sensing units include the use of microneedles, nanoneedles or unconventional sample collection methods (such as face masks) for easy and continuous or on-demand sampling as well as the further integration of micromaterials or nanomaterials and stabilized synthetic biology reactions for signal amplification.
Moreover, novel biorecognition elements or assay technologies (such as aptamers, molecularly imprinted polymers, nanozymes, DNAzymes or CRISPR–Cas-powered assays) could be applied to increase sensitivity and facilitate long-term use.
The accuracy of wearables could be improved through multimodal and/or multiplexed sensing by mounting different transducer types and/or simultaneously measuring different analytes and/or samples on the same platform.
In addition, increased use of cloud or fog computing, data mining and ML for the extremely large datasets produced by wearable sensors would also help to enable more accurate predictions of the physiological status of users.
In this respect, the first prerequisite is to conduct larger prospective cohort studies using wearable sensors to validate their clinical applicability for diagnosis and population-level studies.
Before starting these trials, data collection and its use should be planned by considering their implementation in ML models, for both training, validation and testing of these models.
Moreover, social acceptance of wearable sensors must be ensured by informing users about the advantages and disadvantages and by integrating them into existing wearable devices or application ecosystems (such as smartwatches or wearable glucometers) and health-care services (such as health insurance policies; see Supplementary information for further discussion).
In addition, the integration of wearables into Internet-of-Things applications and the adoption of protocols for data safety and handling (such as blockchain177) with the establishment of an ethical regulatory framework for wearable data networks could further promote their use.
In the foreseeable future, extension of the capabilities of wearables beyond diagnostic sensing through the integration of feedback loops would pave the way for (third-generation) wearable devices for theranostic applications.
Smart bandages, for example, could allow for real-time monitoring of wound healing through pH measurement and, in the case of an infection, treatment by on-demand delivery of antibiotics or anti-inflammatory drugs.
Another trend is to enhance the capabilities of current wearable continuous glucose-monitoring systems to release insulin to the patient in a closed-loop manner.
In the foreseeable future, extension of the capabilities of wearables beyond diagnostic sensing through the integration of feedback loops would pave the way for (third-generation) wearable devices for theranostic applications.
Originally published at https://www.nature.com on July 22, 2022.
About the authors & affiliations:
- H. Ceren Ates,
- Peter Q. Nguyen,
- Laura Gonzalez-Macia,
- Eden Morales-Narváez,
- Firat Güder,
- James J. Collins &
- Can Dincer
1 FIT Freiburg Center for Interactive Materials and Bioinspired Technology, University of Freiburg, Freiburg, Germany.
2 IMTEK — Department of Microsystems Engineering, University of Freiburg, Freiburg, Germany.
3 Wyss Institute for Biologically Inspired Engineering, Harvard University, Boston, MA, USA.
4 Department of Bioengineering, Imperial College London, London, UK.
5 Biophotonic Nanosensors Laboratory, Centro de Investigaciones en Óptica, León, Mexico.
6 Institute of Medical Engineering & Science, Department of Biological Engineering, MIT, Cambridge, MA, USA.
7 Broad Institute of MIT and Harvard, Cambridge, MA, USA.
8 These authors contributed equally: H. Ceren Ates, Peter Q. Nguyen.
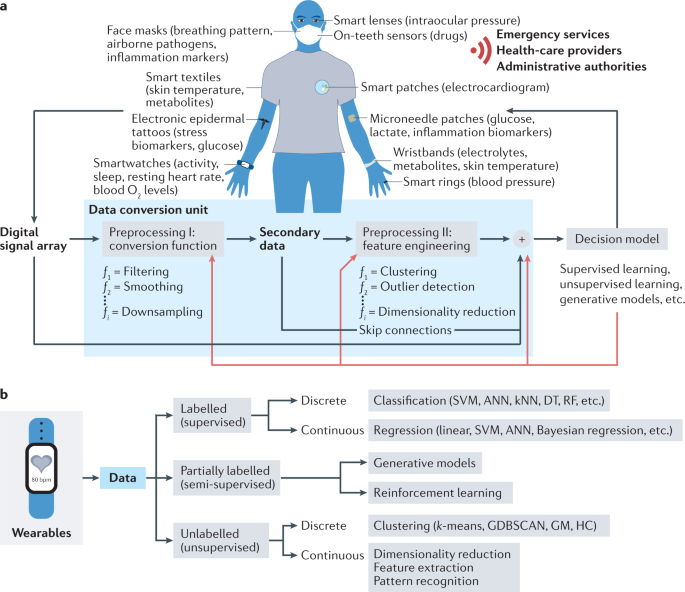